Glossary and abbreviations
3D three-dimensional
ADP adenosine diphosphate
AlkB alkylation B
AMP adenosine monophosphate
ARDS acute respiratory distress syndrome
ATP adenosine triphosphate
BBO Barium Borate
CALI chromophore-assisted light-inactivation
CASSCF complete active space self-consistent field
CDT chemodynamic therapy
CI configuration interaction
CICR calcium-induced calcium release
CISS chirality-induced spin selectivity
cQED cavity QED
CT-QMC continuous time quantum Monte Carlo
DFT density functional theory
DL Delayed Luminesce
DMFT dynamical mean-field theory
DMRG density matrix renormalization group
DNA deoxyribonucleic acid
ECM extracellular matrix
EDT electrodynamic therapy
ELF extremely low frequency
EMCCD Electron-Multiplying Charged Coupled Device
EMF Electromagnetic field
EPR electron paramagnetic resonance
EPTA Entangled two photon absorption
ET electron transfer
ETC electron transport chain
ETF electron transfer flavoprotein
FAD flavin adenine dinucleotide
FDA Food and Drug Administration
FeS iron-sulfur
FMO Fenna–Matthews–Olson
GDP guanosine 5’-diphosphate
GGA generalized gradient approximation
GMF geomagnetic field
GTP guanosine 5’-triphosphate
HER2 human epidermal growth factor receptor 2
HF Hartree-Fock
HIF hypoxia inducible factor
HMF hypomagnetic field
HPD hematoporphyrin derivative
HSP heat shock protein
ICH intracerebral hemorrhage
IR infrared
ISC intersystem crossing
LDA local density approximation
LED light-emitting diode
LHCII light-harvesting complex II
LHN light-harvesting nanotube
LLLT low level light therapy
MCF7* Michigan Cancer Foundation 7
MCF10A* Michigan Cancer Foundation 10A
MFEs magnetic field effects
ML machine learning
MM molecular mechanics
MO molecular orbital
MR multi-reference
MSC mesenchymal stromal cell
NADPH nicotinamide adenine dinucleotide phosphate
NIR near-infrared light
NISQ noisy intermediate-scale quantum
NMR nuclear magnetic resonance
NOX NADPH oxidase
OXPHOS oxidative phosphorylation
PBM photobiomodulation
PCET proton-coupled electron transfer
PDT photodynamic therapy
PEG polyethylene glycol
PEMF pulsed electromagnetic field
PMT Photomultiplier Tube
PSII photosystem II
PTT photothermal therapy
QA quantum annealing
QED quantum electrodynamics
QM quantum mechanical
QM/MM quantum mechanics / molecular mechanics
RDT radiodynamic therapy
RET resonance energy transfer
RIRR ROS-induced ROS release
RNA ribonucleic acid
ROS reactive oxygen species
RPM radical pair mechanism
SCC-DFTB self-consistent charge density-functional tight-binding
SCF self-consistent field
SD Standard deviation
SDT sonodynamic therapy
SFG Sum Frequency Generation
SR stochastic resonance
STM scanning tunneling microscopy
THz terahertz
TRIM tissue resonance interaction method
Trp tryptophan
UPE ultraweak photon emissions
UV ultraviolet
UVR8 UV resistance locus 8
WMF weak magnetic field
With thanks to Nathan Babcock.
Read Nathan's book, Physical Principles of Quantum Biology, here.
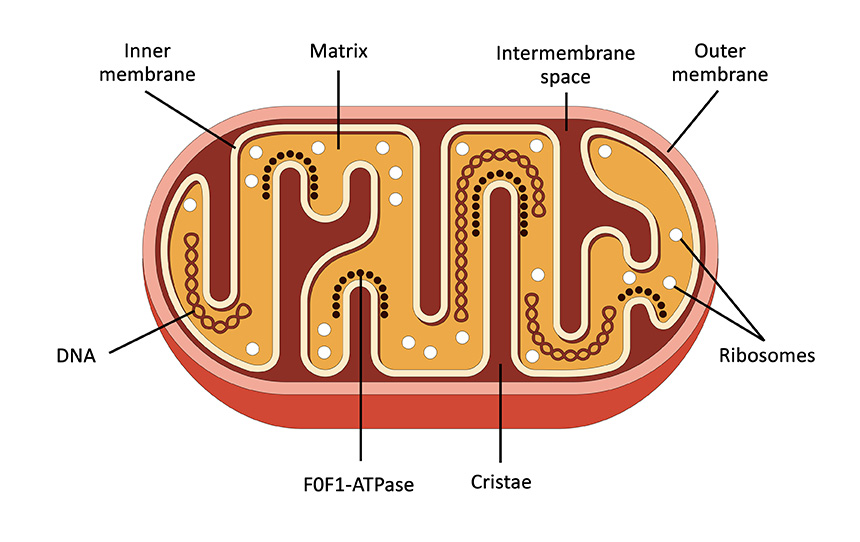